Cosmic rays in star-forming regions
Thanks to new observations from radio
to infrared wavelengths and data collected by the Voyager 1 and 2 spacecrafts,
the role of cosmic rays in star-forming environments has recently received
renewed attention. Theoretical models have shown that low-energy cosmic rays
(<TeV) play a fundamental role in shaping the chemical richness of the
interstellar medium, and in determining the dynamical evolution of molecular
clouds and protostellar discs. These models have also highlighted the
importance of energetic particles accelerated in shocks associated to the star
formation itself (accretion shock, bow shocks, internal jet shocks), the
so-called local cosmic rays.
The origin of chemical complexity in
molecular clouds
Without cosmic rays, molecular clouds would be mainly made up
of molecular hydrogen, helium, and some atomic species ionised in the outer,
more diffuse regions subject to the interstellar ultraviolet radiation field.
The only way to explain the presence of complex molecules, which are routinely
observed, is by invoking the ionisation of H2 by interstellar cosmic
rays, the only agent able to penetrate into the densest parts of molecular
clouds. Figure 1 shows an example of the network of reactions generated by the
ionisation of H2 by cosmic rays. In particular, the hydrolysis of
HCN oligomers leads to the formation of amino acids and DNA nucleotides, with
obvious implications for the origin of life. In addition, secondary electrons
produced by cosmic rays heat the gas in the densest parts of molecular clouds
shielded to UV photons and X rays (Glassgold et al. 2012), and dissociate H2 producing
a residual fraction of atomic hydrogen that controls the hydrogenation process
to form complex molecules such as methanol and acetaldehyde (Padovani et al. 2018b).
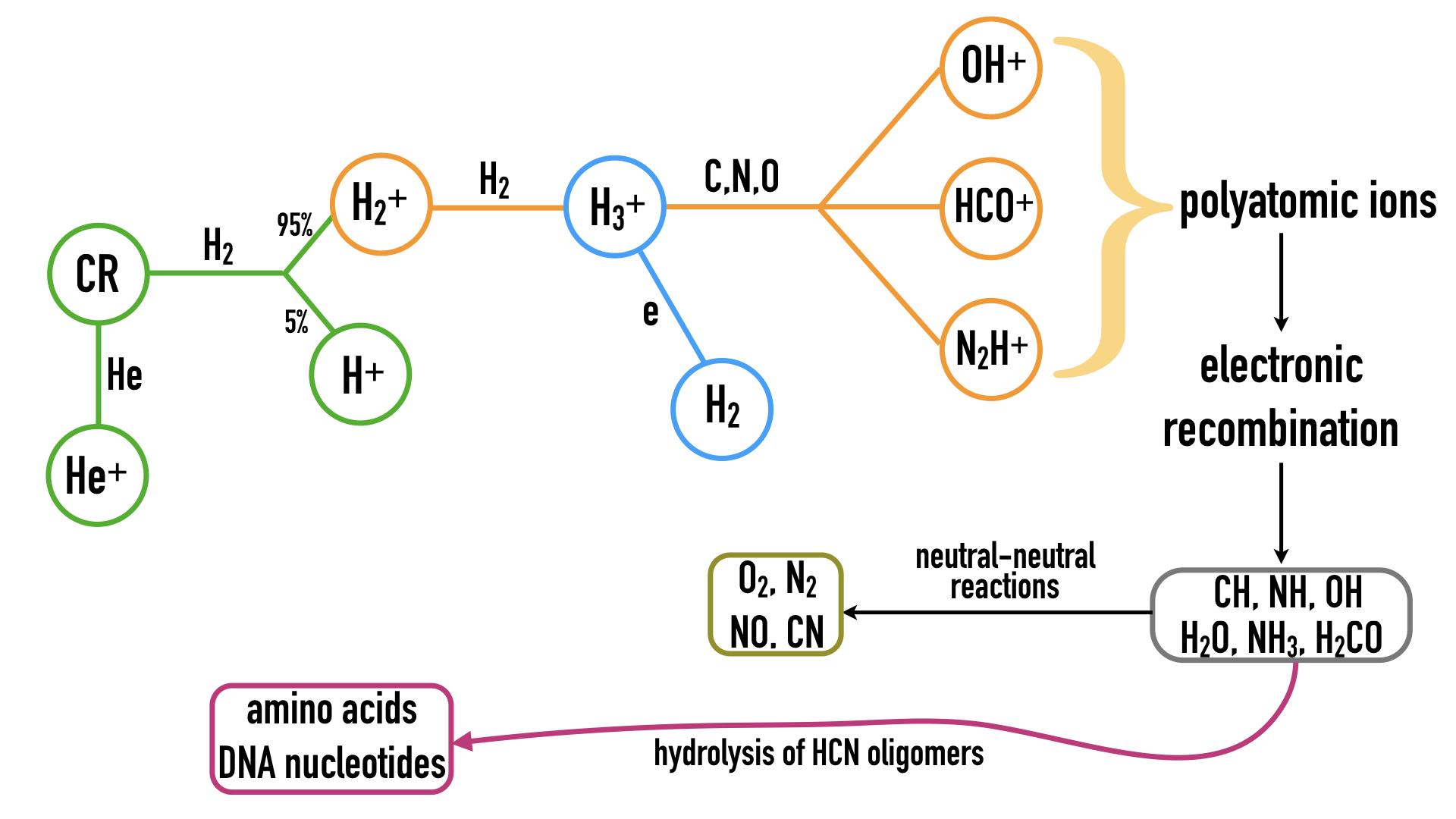
Figure 1. Example of chemical network generated by cosmic
rays (CR) in a molecular cloud. Adapted from Padovani et al. (2014a).
Cosmic rays as regulators of cloud collapse
Cosmic rays play a key role in the process of star formation.
On the scales of prestellar cores (<0.1 pc) turbulent motions and thermal
pressure are negligible and the only effect that can counteract the
gravitational collapse is represented by magnetic fields. While charged
particles remain attached to the magnetic field lines, the neutral component
diffuses towards the centre of the cloud. The friction between charges and
neutrals, called ambipolar diffusion, modifies the geometry of the magnetic
field, creating typical hourglass configurations (Beltrán et al. 2019) and slows down the collapse
of the neutral component due to collisions with the charges. The effectiveness
of this process depends on the degree of coupling between the gas and the
magnetic field which is proportional to the ionisation fraction of the gas
that, in turn, is determined by the ionisation induced by cosmic rays.
Our team has analysed in various studies the
attenuation of the cosmic-ray flux during its propagation inside a cloud and
the consequent decrease of the ionisation rate ζ (number of H2
ionisation per unit time) with increasing column density (Fig. 2).
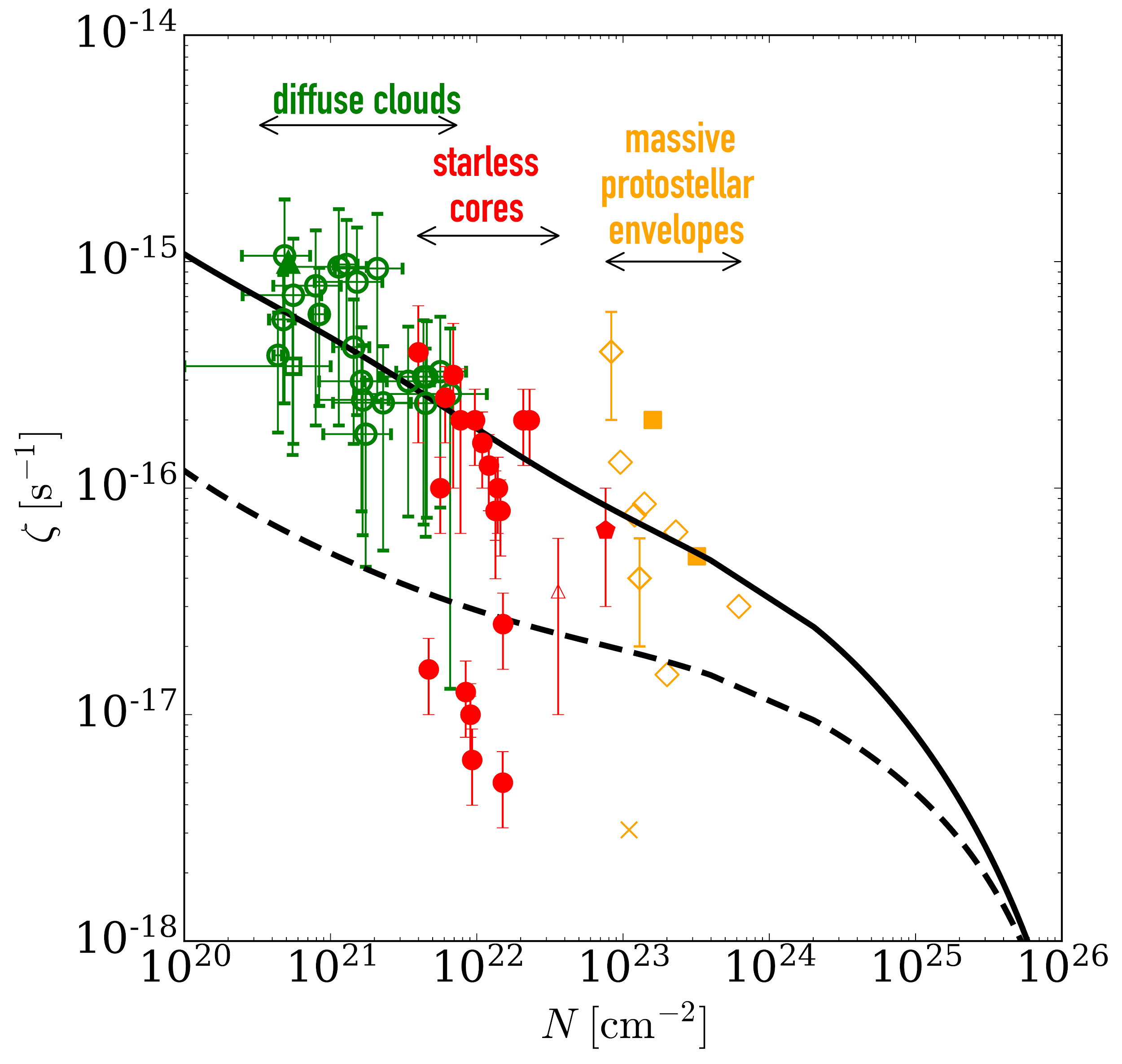
Figure 2. Cosmic-ray ionisation rate versus H2
column density. The dashed and solid black lines represent the model based on
Voyager data and on an enhanced flux at low energy, respectively. Observations
(coloured symbols) are better explained by the latter model. See Padovani et al. (2009); Padovani & Galli (2011); Padovani et al. (2013, 2014b); Padovani et al. (2018a, 2020), for details.
Locally accelerated
cosmic rays
In recent years, a number of
observational studies revealed an extremely high value of ζ in
star-forming regions (e.g., Ceccarelli et al. 2014; Podio et al. 2014; Fontani et al. 2017). In addition, synchrotron
emission, an indication of the presence of relativistic electrons, has been
recently observed in the bow shock and in the knots along low- and high-mass
protostellar jets and in HII regions (e.g., Sanna et al. 2019; Meng et al. 2019; see Fig. 3). Since
protostars are located in the innermost and highly shielded region of molecular
clouds, the high levels of ionisation and the synchrotron emission cannot be
ascribed to Galactic cosmic rays. A new theoretical model (developed in Padovani et al. 2015, 2016, 2019) shows how thermal charged
particles can be locally accelerated and transformed into cosmic rays in jet
shocks or directly on the surface of a protostar. The model is based on the
first order Fermi acceleration process, also invoked to explain the origin of
high-energy cosmic rays in supernova remnants. The model is able to explain the
available GMRT, EVLA, IRAM-30m, NOEMA, and Herschel observations.
The importance of having identified
an internal source of cosmic rays in a protostellar system has a strong impact
on the theory of planetary formation. If local cosmic rays are able to reach
the disc, they can change its ionisation degree with consequences on the formation
rate of complex and prebiotic molecules and the efficiency of planetesimal
formation (see also Ivlev et al. 2015). In fact, on the one hand
cosmic rays increase the mobility of simple molecules on the surface of dust
grains, favouring their combination into complex molecules, on the other hand
the more energetic and penetrating cosmic rays can change the extension of the
so-called dead zone, reactivating the magnetorotational instability, modifying
the planetesimal and planet formation efficiency.
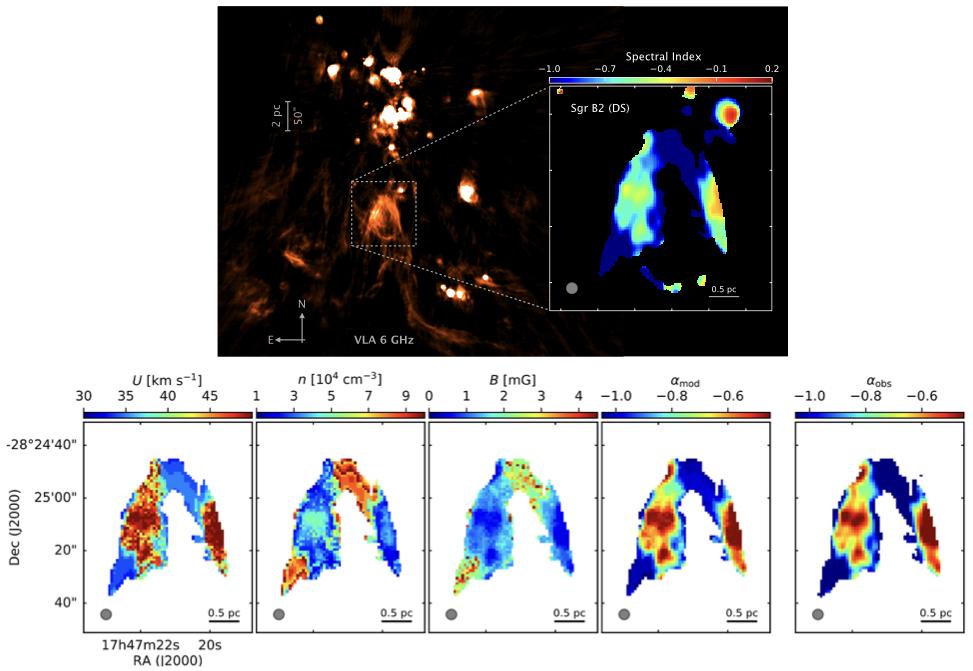
Figure 3. Upper
panel: VLA observations of the region Sgr B2. The inset shows a zoom in the
region so called <<Deep South>> (DS) where the spectral index has
been computed. Lower panels: Maps of shock velocity (U), volume density (n),
and magnetic field strength (B) of DS that reproduce the observed flux density
maps obtained by the model described in Padovani et al. (2019). The model also generates the spectral index map (αmod) which is consistent with the observed αobs map (from Meng et al. 2019).
Future projects and
perspectives
The study of low-energy cosmic rays
opened a new line of research, allowing a strong synergy between theoretical
models, observations, and laboratory experiments. In addition, the acceleration
mechanism of local cosmic rays configures a number of interconnections with
other areas:
- Stellar cosmic rays are necessary
to explain the formation of complex molecules on dust grains. Laboratory
studies of ice irradiation with ionised particles will be useful to verify the
possible formation of prebiotic molecules;
- The theory foresees that cosmic
rays can be accelerated up to ~1 TeV in knots of high mass protostellar jets,
with a spatially limited, but not negligible 𝛾 emission. Feasibility
studies need to be performed to understand if
CTA will be able to separate this local
contribution from the Galactic background. This is not possible with current
instruments like H.E.S.S. and Fermi, because of their low resolution;
- A further development is the
effect of stellar cosmic rays, generated, e.g., in red dwarf flares, on
exoplanetary atmospheres. In fact, stellar cosmic rays influence the ozone
abundance in the atmosphere. Stratospheric O3 is determinant for the
UVB flux that can reach the surface of the planet, conditioning the presence of
life forms. Telescopes such as
E-ELT/HIRES, ARIEL, and JWST will give information on the abundance of biological tracers
(O, O3, NO2) in exoplanetary atmospheres that will be
compared with the predictions of theoretical models. In this way it will be
possible to better characterise the probability of habitability of a planet;
- Understanding the role of
magnetic fields in star-forming regions is of fundamental importance. In the
near future, the exceptional sensitivity of
SKA will offer a unique opportunity to determine the magnetic
field strength in molecular clouds through synchrotron emission observations (Padovani et al. 2018c). Precursors of SKA like MeerKat and ASKAP, as well as E-LOFAR will provide a substantial
contribution to a first characterisation of Galactic synchrotron sources.